Gestalt-Energy
The conglomerate through its Gestalt-Energy subsidiary takes a strategic position in R&D for nuclear energy that is generated through molten salt reactors that uses thorium as the fuel. This represents the future of unlimited, safe, and inexpensive energy.
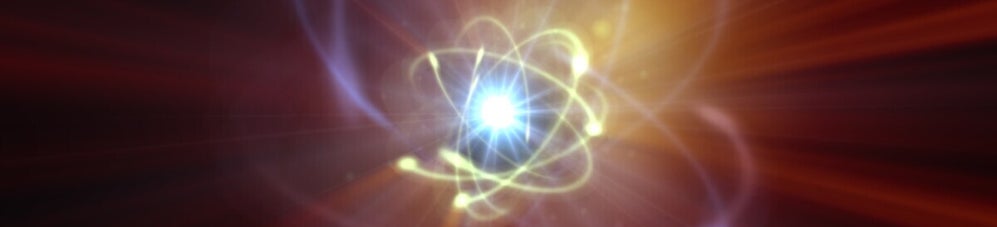
Gestalt Energy is primarily engaged in Research and Development of Lithium Fluoride Thorium Reactors (LFTR), batteries, solar, algae bio-fuels and micro-grid systems.
Pomp and Circumstance March
Lithium Fluoride Thorium Reactor ( LFTR)
LFTR is expected to become the major energy source for most of the world. A great amount of attention has focused on LFTR as the major energy source for the world. LFTR is a specific type of Molten Salt Reactors (MSR) that was discovered and operated in the 1960s by the US government. A brief introduction to MSR and LFTR is provided to understand the enormous potential LFTR offers as a clean abundant source of energy and its byproducts needed for medical treatments. LFTR use molten fluoride salts for the coolant.
LFTR operates at low pressure, since they do not need high pressure to transfer heat from the reactor to the generator. Thorium is the major fuel source used by LFTR. It is dissolved in a liquid Lithium-Beryllium-Fluroide (LiF-Be-F2) salt that remains in a liquid state without pressure from 500o C to 1400o C. Whereas most nuclear reactor plants are high Pressure Water Reactors (PWR) that operate at 315o C and operate at 150 atmospheres pressure. This characteristic requires enormous infra-structure to contain a leak if one should occur.
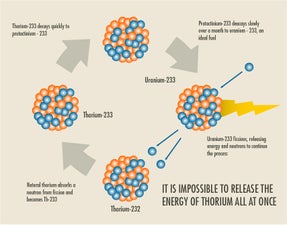
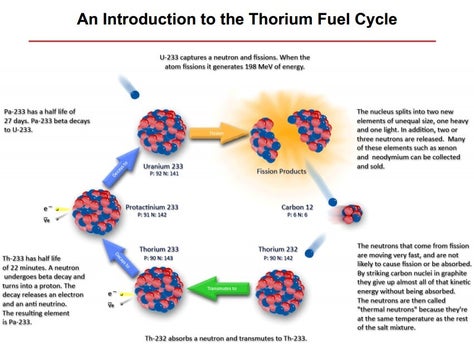
LFTR use dissolved fuel in the salt as the coolant which is easily reprocessed in the system. All the essential nuclear elements that include Thorium, uranium and plutonium can form fluoride salts that dissolve in the LiF-Be-F2 (FLiBe). This enables separation from the fluoride form. Graphite is used as a moderator with the molten salts. This is essential to allow fission in the LFTR to product the heat necessary to generate electrical power.
Energy
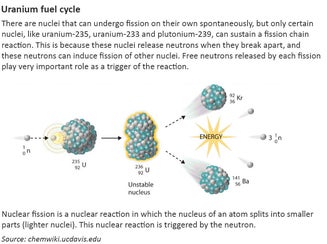
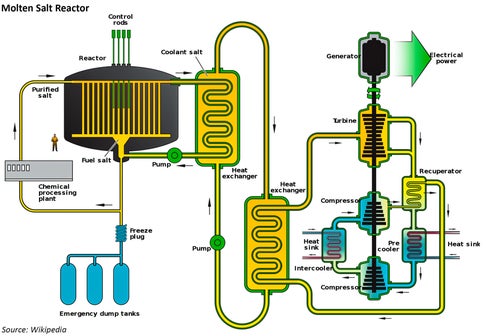
Fluoride salt mixtures have many attractive features that recommend them for use in a nuclear reactor. They are very chemically stable and impervious to radiation damage due to their ionic bonding. Although they do not melt until operated at elevated temperatures (>350C) they have a wide liquid range beyond their melting point, approximately a thousand degrees Celsius. They dissolve useful quantities of actinide fluorides such as uranium tetrafluoride, thorium tetrafluoride, and plutonium trifluoride. They chemically capture fission products such as cesium and strontium in fluoride form and prevent their release. Most importantly, they operate at high temperatures yet at essentially ambient pressures, removing concerns about pressurized reactor operation.
All I Have To Do Is Dream - Everly Brothers
Fluoride salt mixtures also have excellent volumetric heat capacity, somewhat better even than water. The volumetric heat capacity of the coolant is the basic yardstick that sizes a reactor and the rate at which its coolant must be pumped, giving fluoride salt reactors a great advantage over other designs.
Fluoride salt mixtures also have excellent volumetric heat capacity, somewhat better even than water. The volumetric heat capacity of the coolant is the basic yardstick that sizes a reactor and the rate at which its coolant must be pumped, giving fluoride salt reactors a great advantage over other designs.
Annie's Song - John Denver
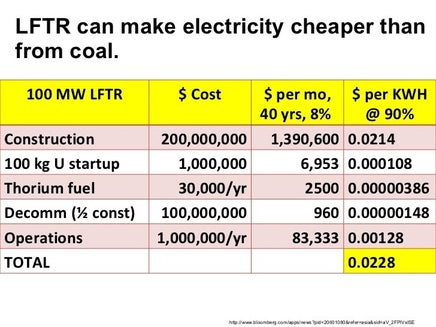
For uranium reactors, natural uranium is mined, purified, and chemically converted to uranium hexafluoride prior to enrichment. The process of enrichment results in two output streams, one enriched and one depleted. Approximately five parts out of six of the original uranium ends up in the depleted stream, with only one part out of six going on to be fabricated into nuclear fuel. Most of the small amount (0.7%) of the original uranium that is uranium-235 ends up in the enriched stream, but about a third remains in the depleted stream. The enriched uranium hexafluoride is chemically converted back to uranium dioxide, pressed into pellets, loaded into zirconium tubes to form fuel rods, and arranged into clusters to form fuel assemblies. These assemblies are then loaded into a reactor where they will spend approximately five years in the core in various locations to generate nuclear energy. At the end of their useful life, they are removed from the reactor and allowed to cool in a spent fuel pond.
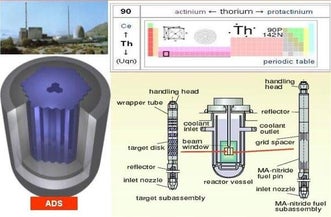
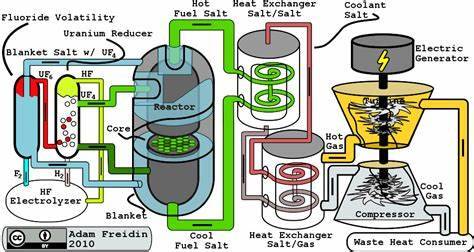
Most of their uranium-235 has been consumed. Some of their uranium-238 has been converted into plutonium-239 and some of that has also been consumed. Fission products have been generated from the fission of both uranium-235 and plutonium-239. Several long-lived isotopes of plutonium, americium, and curium have been formed when plutonium-239 absorbed a neutron rather than fissioned. These long-lived actinides present a disposal challenge, yet their formation at a rate that exceeds their consumption is inevitable when uranium fuel is used in a thermal-spectrum reactor of any type, including a molten-salt reactor.
Somewhere over the rainbow
In a future liquid-fluoride thorium reactor, the fuel cycle would be quite different. The reactor would ideally be started by a modest inventory of uranium-233. Fission of U-233 in the reactor generates thermal power as well as excess neutrons that would be captured in a blanket fluid containing thorium tetrafluoride in solution. Thorium, having absorbed a neutron, first decays to protactinium and ultimately to uranium-233. New fuel would be chemically removed from the blanket fluid either at the uranium stage or the protactinium stage, which has additional complexity and advantages. The new uranium fuel would be introduced into the fuel salt of the LFTR at the same rate at which it is consumed.
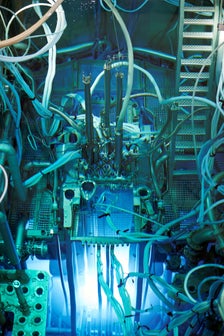
Uranium-233 is consumed at high efficiency (91%) in a thermal spectrum reactor and that which is not consumed goes on to form uranium-235, which is also consumed at high efficiency (85%) in a thermal spectrum reactor. This limits considerably the amount of material that can reach the stage of the first transuranic, in this case, neptunium-237, and thus the issue of long-lived actinide waste production. The fuel salt used in the LFTR is chemically processed as the reactor operates, removing fission products while retaining actinide fuels. This allows the creation of an actinide-free waste stream which decays to acceptable radioactivity levels in approximately 300 years, strongly governed by the 30-year half-lives of cesium-137 and strontium-90.
Bring Him Home Safely To Me - Sandy Posey
Thorium fuel used in a LFTR has the potential to achieve approximately 200 times the fuel efficiency as uranium fuel used in existing reactors. In addition, the LFTR operates at high temperatures and this makes possible the implementation of power conversion systems based on supercritical carbon dioxide gas that can achieve thermal efficiencies of approximately 45%, meaning less of the thermal power generated by the reactor need be rejected to the environment, and more can be converted to electrical energy for the consumer.
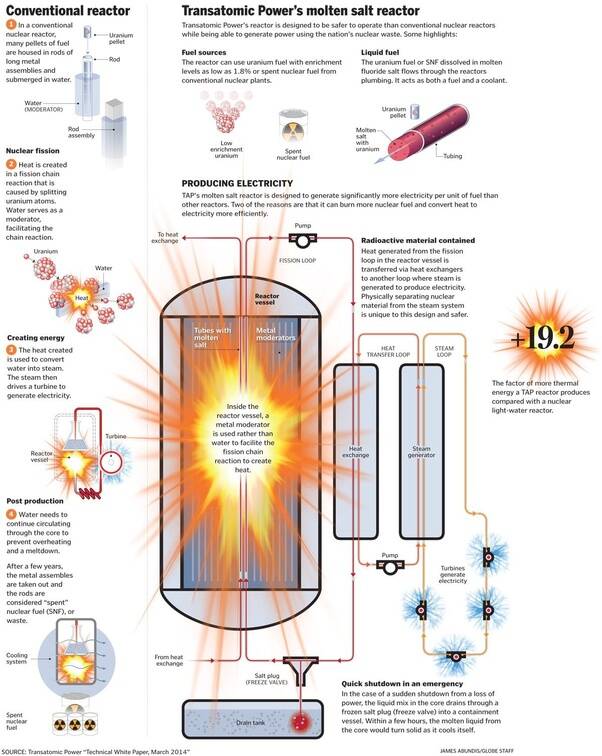
The high operating temperatures of the LFTR also enable direct applications of its process heat to be considered, such as the thermochemical generation of hydrogen, which could become an important part of the transportation infrastructure of the country in the future.
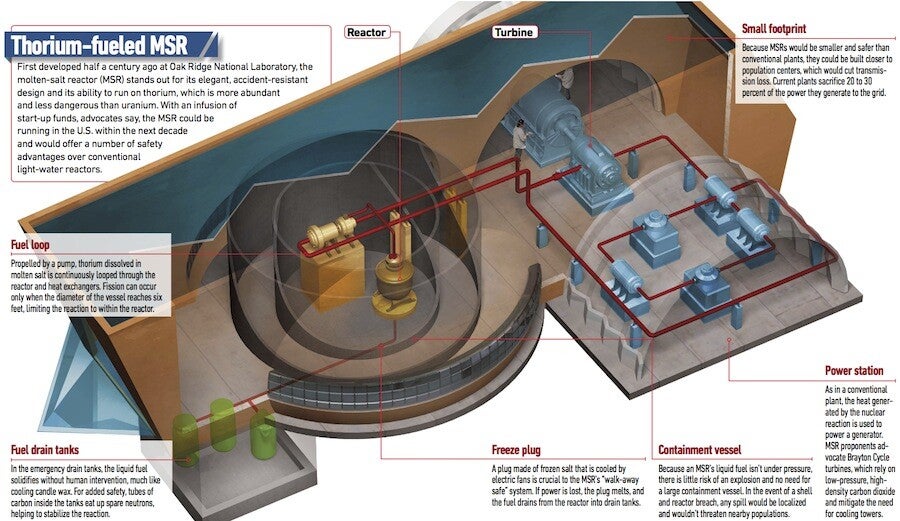
Nuclear fission also produces other valuable materials as the products of fission, most notably several important medical radioisotopes. Each of these products is characterized by a rather short half-life, which means that existing solid-fueled reactors cannot extract them quickly enough before their value is lost to decay. Currently these isotopes are produced in dedicated materials testing reactors in Canada and the Netherlands. Unfortunately each of these reactors is scheduled to be permanently shut down next year, putting world supply at risk. The LFTR not only produces these isotopes but its fluid fuel means that they can be readily extracted. This can stabilize world supply and provide an additional source of revenue for the reactor’s operator.
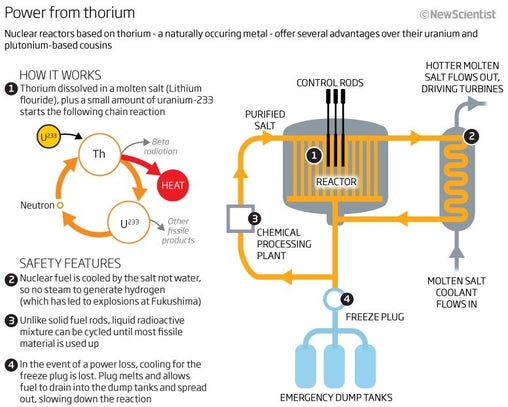
Through the realization of additional revenue through products like hydrogen, process heat, medical radioisotopes, and even desalinated seawater, the levelized cost of electricity that would need to be charged by a reactor’s operator could potentially be reduced.
The fluoride chemical form of the fuel is another important advantage, chemically trapping fission products such as cesium, strontium, and iodine in chemically stable forms and strongly limiting their potential for dispersal. The fact that the salt is only fluid at high temperatures is another advantage for reactor safety, meaning that if it is cooled to ambient temperatures it freezes and traps fission products therein.
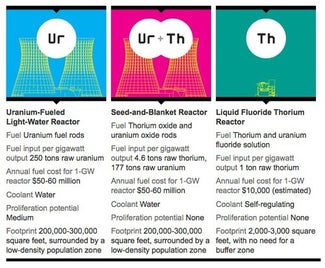
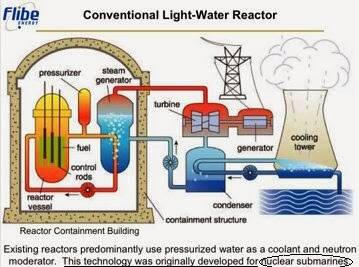
There are significant challenges that remain before LFTRs can be deployed in the scale necessary. The high-nickel alloy proposed for use with the reactor (Hastelloy-N) must be ASME code-qualified to permit certified construction. Suitable core designs that achieve safety and neutronic goals must be refined and tested. The carbon dioxide power conversion system must be scaled up and demonstrated. Capture of tritium formed from neutron absorption in lithium salt must be demonstrated to satisfaction. Suitable heat exchanger designs for a variety of locations in the reactor must be completed, tested, and proven.
The chemical processing system that enables the thorium fuel cycle must be demonstrated at a prototype scale, and then at a larger bench scale, before ultimately being tested in a real reactor. Associated with this will be the need to demonstrate that long-lived actinides can be excluded from the waste stream through the proper operation of the chemical processing system. And perhaps most importantly, a business case that accounts for the totality of potential products from the reactor must be developed and shown to be competitive with low-cost hydrocarbon fuels.
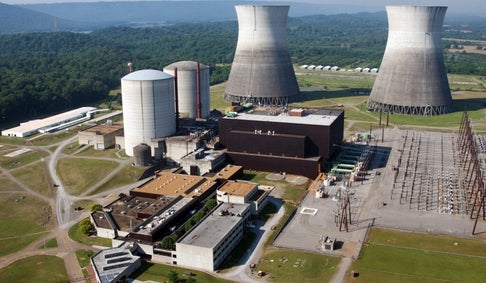
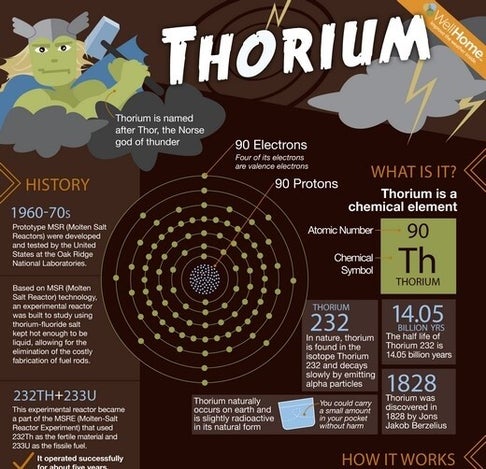
Producing a gigawatt of electricity for a year would only consume less than a tonne of thorium fuel, and the United States has 3200 metric tonnes of thorium in easily accessible disposal areas, in addition to hundreds of thousands of tonnes in geologic deposits like the Lemhi Pass area of Idaho. If used efficiently in a LFTR, thorium could provide energy security for the United States.
All I Have To Do Is Dream - Everly Brothers
Micro-grid
Gestalt Energy is also engaged R&D, design, manufacture and business planning for micro-grid systems. The micro-grid is a self-sufficient energy system that serves a limited geographic area. The micro-grid produces its own power using distributed power produced from sources like solar, wind turbines, small nuclear reactors and other sources. Recently micro-grids include batteries for energy storage. A micro-grid provides electricity that is controlled by software. Micro-grids are different from the large central grids that create electric power from plants that are distributed through transmission and distribution lines over large distances. Central power that is delivered over long distances results in the loss of 8% to 15% of the power that dissipates over the transmission lines.
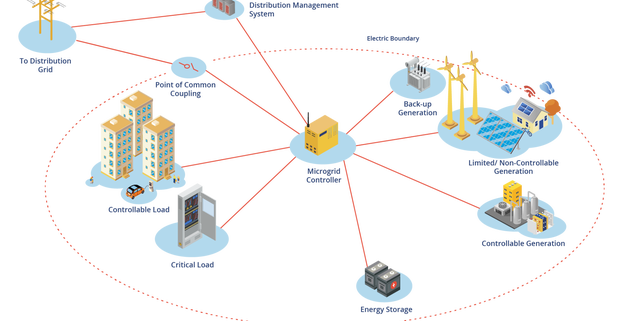
Microgrids are small groupings of interconnected power generation and control technologies that can operate within or independent of a central grid, mitigating disturbances and increasing system reliability. By enabling the integration of distributed resources such as wind and solar, these systems can be more flexible than traditional grids. This market presents a new and important opportunity for end users, generators, and planners to provide increased efficiency and resiliency in the evolving grid.
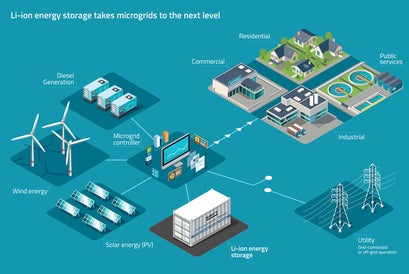
Distributed energy resources allow electricity to be generated closer to where it is used, protecting businesses and institutions from unexpected outages caused by natural disasters and other disruptions. The U.S. national laboratories as well as public-private partnerships provide financial resources and access to research facilities to foster innovations to modernize the power sector from a 100-year-old centralized system to one that incorporates disparate clean technologies such as microgrids, batteries, and energy smart tools. These investments and the resulting new products and capabilities decrease costs, improve grid reliability, reduce emissions, and offer consumers more options.
The Wind Beneath My Wings - Gary Morris
Since micro-grids are close to customers, there is very little power loss over distribution lines. Micro-grids are not going to replace central power and its transmission and distribution line. But micro-grids are a good source of power when the central power grid experiences a failure, such as from a storm. Modern micro-grids have a controller that functions as the brain for the system and orchestrates the multiple energy resources needed when there is a failure in the central grid. Where there is no central power grid available, the micro-grid is an excellent source for power, such as for a military base camp.
The loss of grid power can mean major interruption to mission-critical work that may have catastrophic results to your business. As organizations are becoming increasingly concerned about reliable utility supply, power quality and the vulnerability of the grid, many are taking greater responsibility to secure their own energy supply and infrastructure – particularly those organizations and industries dependent on reliable power without interruption.
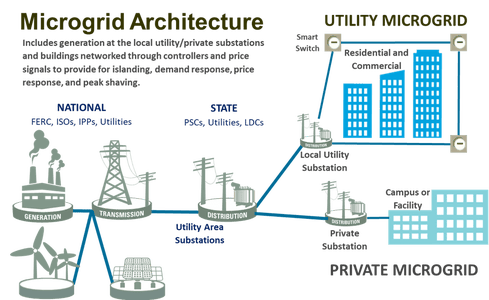
To create a more robust, resilient and secure energy infrastructures, Distributed Energy Resources (DER) can provide reliable solutions not only in driving greater energy efficiencies and insuring critical resources, but also as viable alternatives to traditional centralized generation.
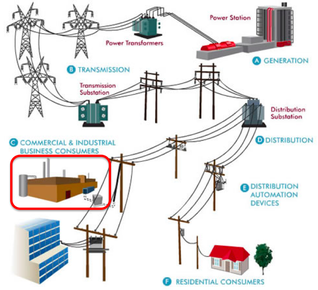
In addition to providing built-in resilience and business continuity, microgrids offer several other benefits. They can synchronize and connect to the grid during normal conditions, while also providing the capability to disconnect and operate independently as an “island” if needed. At certain times, utility energy costs might be high enough that it becomes cheaper for a facility to generate power itself.
Using renewables to displace grid energy can provide sustainability benefits. And finally, power quality issues from the local utility could occur, and a microgrid could mitigate those specific supply problems before they impact the consumer.
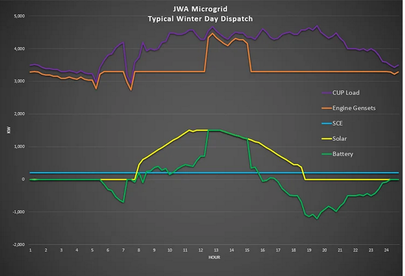
When designing a microgrid the most used option is to use diesel generators to fill the power gap. A better approach is to have a microgrid solution the fills the gap between fossil fuel carbon emissions and sustainability.
Solar and battery combinations are not a solution for energy resiliency for commercial and industrial (C&I) customers with office and retail spaces in high-rise buildings or data centers in cities. Solar simply can’t deliver the necessary energy intensity given the space limitations. Fuel cells can play a major role serving these scenarios.
This points to the need to have a solution with greater power density, such as fuel cells, for community-level resiliency from microgrids. However, it’s not a “one or the other” problem. Because fuel cells integrate seamlessly with advanced microgrids and renewable DERs, their deployment can be right-sized with solar and battery systems, depending on needs
Stationary fuel cells can run on natural gas, which is readily available and cleaner than the diesel used by diesel generators, and they are 1.5-2 times more efficient than diesel generators. Where sources of green hydrogen and renewable biogas are available, fuel cells are already using these fuels to deliver electricity with even lower carbon emissions. Importantly, the efficiency remains high even as different kinds of fuel sources are blended into the mix or replace natural gas entirely.
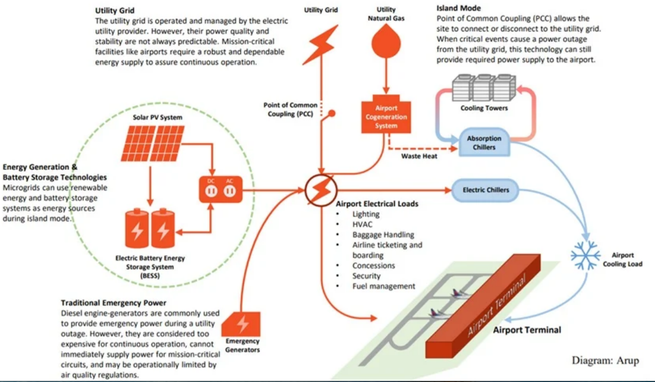
Solar PV combined with battery storage microgrids are ascending as a new zero-carbon reliability solution of choice. However, they are not the Holy Grail for all situations. Many customers operate in a low sunlight region or space-limited facility in which a solar and battery storage-based microgrid won’t deliver the power needed.
The space constraint must be considered when designing a microgrid. For example, a 1-MW solar PV system in a region with adequate solar resources would require a surface the size of three football fields with direct access to sunlight. The same 1 MW from fuel cells would require about 20% of a single end zone and can be installed almost anywhere, such as in basements or underutilized buildings. A solar MW and a fuel cell MW are not created equal either. One MW of installed solar capacity produces about 1.5 MWh of electricity per year. One MW of solid oxide fuel cell capacity generates about 8.8 MWh.
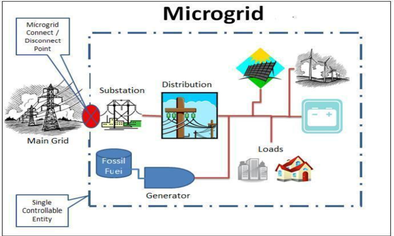
Fuel flexibility of fuel cells is a critical advantage to fill the sustainability gap that stands between the power solutions of today and net zero. Cleaner than today’s diesel generators running on natural gas, sources of low- and no-carbon hydrogen and renewable biogas can be mixed into the natural gas supply to the fuel cell with marginal impact on performance as supply of those fuels ramps up. The fuel cells will remain highly efficient, providing electricity and heat that gets cleaner as the fuel mix transitions. Ideally, the fuel supplied to the fuel cell will transition to zero-carbon hydrogen, making the system truly emissions-free.
Gestalt Energy is engaged in LFTR and micro-grid R&D. It also provides data science, artificial intelligence / machine learning, modeling , chemical and electrical engineering and energy consulting services for oil & gas and chemical processing business segments.
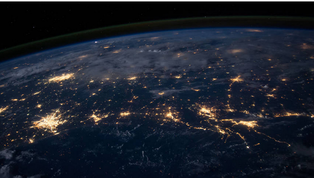
Algae Biofuel
Algae can produce biofuel. Algae are cultivated in large pools or farms. The micro-organisms convert sunlight to energy, and store the energy as oil. The oil is extracted using a mechanical process such as pressing or using sound waves, or with chemical solvents that break down the cell walls and release the oil.N
Algae are among the simplest organic producers in the world, using light and carbon dioxide to make biomass. Green algae, for example, use photosynthesis to grow. But algae aren’t only classed as plants. Rather, they’re a highly varied and genetically diverse group of organisms hailing from across four biological kingdoms: Bacteria, Chromista, Plantae, and Protozoa.
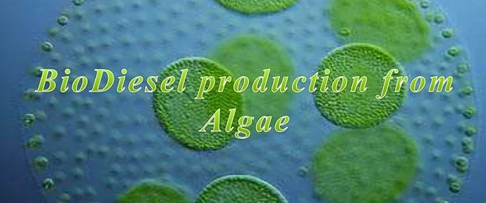
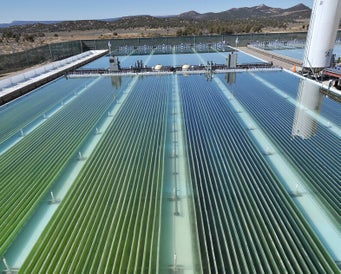
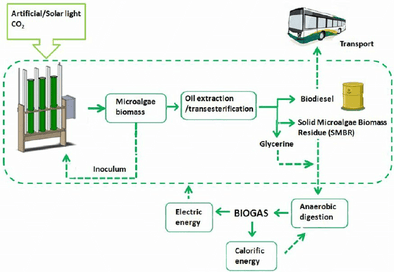
Algae are single-celled organisms that grow in water and are one of the most efficient producers of plant oils. Certain types of algae contain natural oils that can be distilled into a number of petroleum-like products that could act as replacements for gasoline, diesel, and jet fuel.
Using algae for fuel was first explored in the 1970s. The surge in recent interest for renewable, domestically-produced fuels has arisen from worries that the future demand for fuels will outstrip the supply, and that we need to find low-carbon alternatives to petroleum fuels to address the buildup of greenhouse gases, and national security concerns that strongly encourage finding domestic, renewable, and sustainable energy sources.
There are economic and environmental advantages of using algae as biofuel, but there are some disadvantages as well. If we are going to have any sort of mass adoption and use of algae biofuel, it’s important to understand both the pros and cons. Algae are cultivated in large pools or farms to produce biofuel.. The micro-organisms convert sunlight to energy, and store the energy as oil. The oil is extracted using a mechanical process such as pressing or using sound waves, or with chemical solvents that break down the cell walls and release the oil.
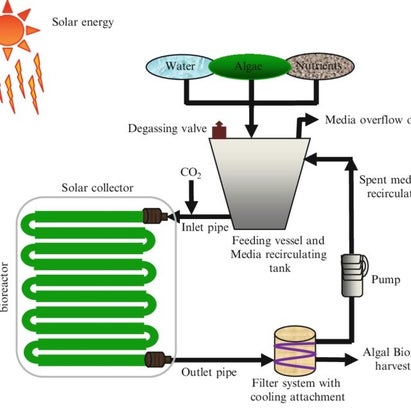
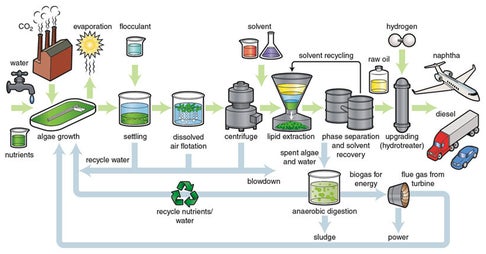
Algae cost more per unit mass than other second-generation biofuel crops due to high capital and operating costs, but are claimed to yield between 10 and 100 times more fuel per unit area. ... This is less than 1⁄7 the area of corn harvested in the United States in 2000.
Algae for biofuels is typically farmed in ponds, using the sun. Hann's method is being used grow the algae in a confined space, just using electricity from the solar panels. This method uses a bioreactor to grow algae using sunlight.
But the byproducts and scrap from algae can be easily buried as composting. Algae biofuels research and development is needed to discover plausibly breakthroughs, in both biology and engineering.
Biofuel is fuel derived from biological material that was recently living, such as trees, agricultural wastes, grass, or crops. This material is also called biomass, which is simply an organic matter that can be converted to fuel and is regarded as a potential energy source. Biofuel can be solid, liquid, or gas, and is a substitute for traditional fossil fuels like petroleum, natural gas, propane, and coal.
As an alternative to fossil fuels, biofuels can reduce greenhouse gas emissions and increase energy security. They are growing in global use, with industries expanding in North and South America, Europe, and Asia.
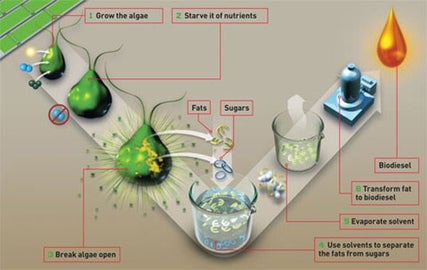
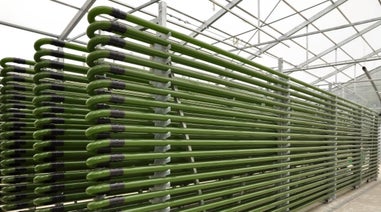
It seems likely that there are over one million algae species. The diversity of algae include from microscopic diatoms to giant sea kelp that grows to 30 meters. Algae contain the building blocks of all organic life: proteins, lipids, carbohydrates and nucleic acids — with lipids especially and potentially useful in energy production. Algae single-celled marine organisms produce more than 20% of the earth’s oxygen.
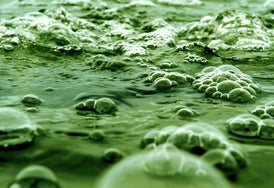
Making algae into biofuel starts in a lab, where each strain is tested and then modified genetically to grow faster and be richer in lipids, the non-water-soluble fatty acids that produce algae oil, the essential ingredient of the hoped-for liquid biofuel. Microalgae can contain 15 to 77% oil, making it an attractive candidate for biodiesel fuel production.
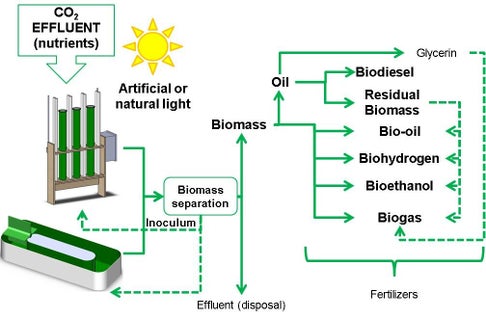
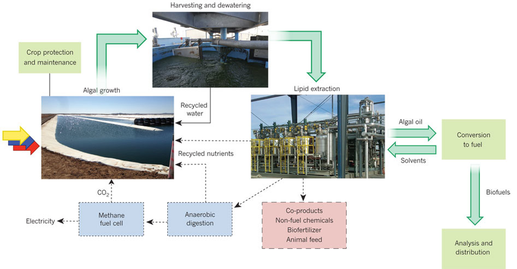
Bioprospecting is the process of finding, testing and finessing algae strains to grow stronger and faster. The objective is to grow and develop lipid rich algae in the lab that would create the most productive microalgae.
T
he algae must be harvest and break down the cell walls with chemical solvents, then extract the inner lipids, proteins and carbs, which undergo a final processing step to convert them into biofuel. Then there is the need to scale up the entire process, growing the microalgae at an industrial scale in vast outdoor pools requiring huge amounts of land and tremendous quantities of fresh or salt water.
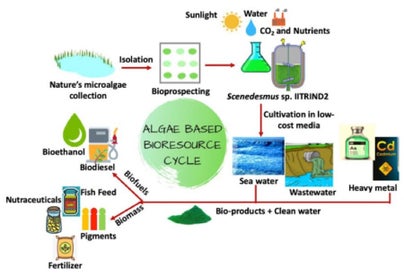
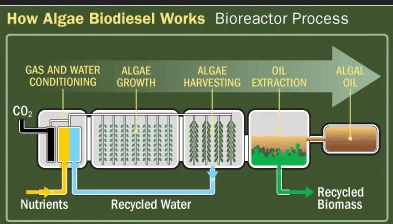
This complexity explains why the algae biofuel industry today continues to be a specialized and very expensive game, only played by those who can afford and tolerate high risk. There are not many companies involved in the algae biofuel business segment because the science is a lot tougher than initially thought. Even with gene editing such as CRISPR technology, it will likely take a large investments to make the algae biofuel science commercially work.
Gestalt Energy’s objective is to develop algae strains that product biofuels that are cost-competitive with fossil fuels. The company’s scientist are tailoring algae cultivation and discovering methods to make algae grow faster in large outdoors ponds. The scientist have used CRISPR gene-editing technology to create algae strains that have been shown to have the capability to become the ultimate energy-producing microalgae strains.
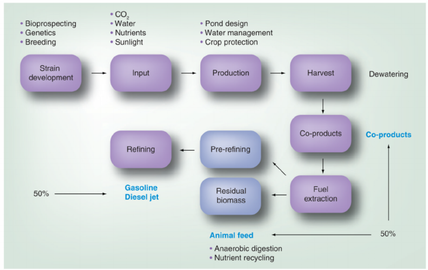
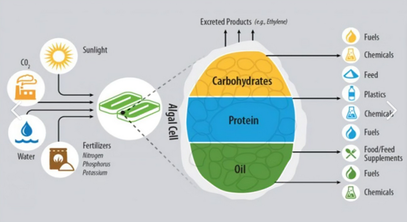
The development of a commercial algae biofuel business is strategic. Avant-Garde-Technologies Corp will continue to spend adequate resources to enable algae biofuel to become a cost-effective replacement for fossil fuels and a source for food and fertilizer.
Algae cost more per unit mass than other second-generation biofuel crops due to high capital and operating costs, but are claimed to yield between 10 and 100 times more fuel per unit area. This is less than 1⁄7 the area of corn harvested.
Algae for biofuels is typically farmed in ponds, using the sun. This method can potentially grow the algae in a confined space, just using electricity from the solar panels. A bioreactor is also way to grow algae, typically using light.
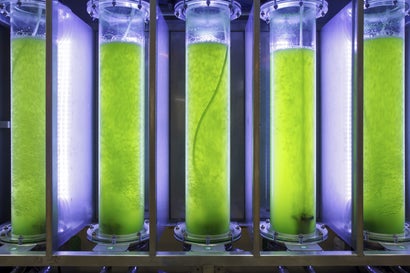
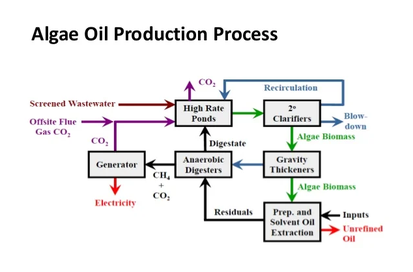
There are also chemical and biological methods, including: 1) solvent extraction (single solvent, co-solvent, and direct reaction by transesterification), 2) supercritical fluids, and 3) enzymatic extraction.: Cyanobacteria called blue-green algae can produce oil from water and carbon dioxide with the help of light
Avant-Garde-Technologies will continue to improve the production processes for algae biofuel. It has committed substantial resources toward continuing R&D in this business, because the potential growth is enormous.
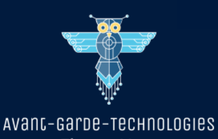